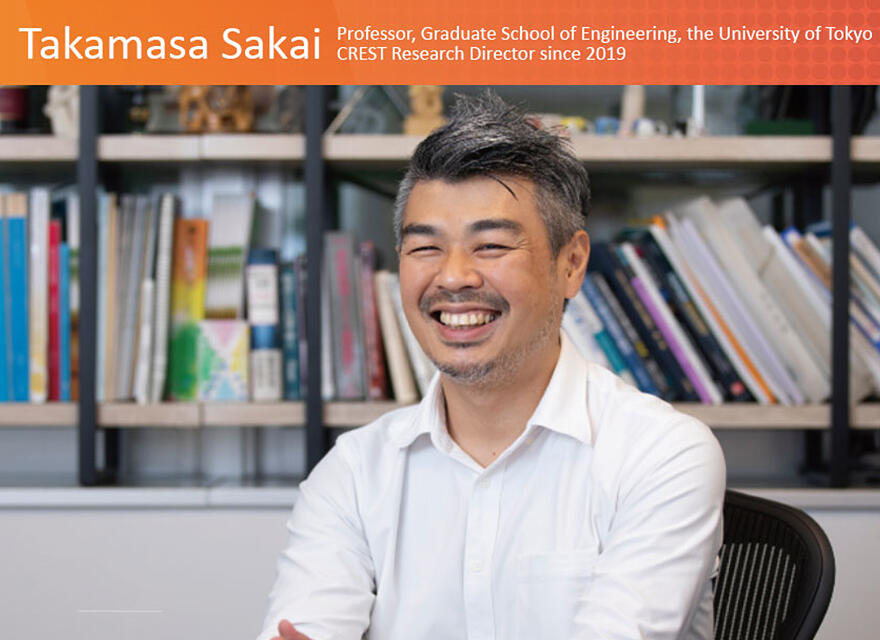
Hydrogels are attracting a great deal of focus as biomaterials thanks to their high compatibility with the human body. They have been put to use as soft contact lenses, among other examples, but as they are dynamically fragile, gels with both strength and durability are needed. Professor Takamasa Sakai of the Graduate School of Engineering, the University of Tokyo, is discovering physical laws that overturn conventional concepts, one after another, as he explores the properties of gels starting from the fundamentals. Based on these scientific principles, he is also taking on the challenge of developing a robust, tough gel that can function as a material for artificial tendons and ligaments.
Materials with plenty of stretch and elasticity - Aiming for co-creation through heterogenous groups
Jelly (jello), konnyaku (konjac) and yogurt are quintessential examples of gels, which are familiar substances to most people. It's well-known that when making jelly (jello), it undergoes a solidification when the hot solution cools. In definition, a gel is a solid and formed from colloid solution (in which polymers and minute particles have dissolved and dispersed in solution, respectively). Polymers and colloidal particles create a 3D network structure that envelopes and confines liquid such as water. Depending on the degree of network formation, the viscous liquid-state sol transforms into a solid gel, representing unique characteristics such as large stretchability and elasticity.
Researchers are making use of these characteristics, utilizing polymer gels in a wide range of fields, from food, farming, medicine to space science. Among them, hydrogels, which contain water as solvent, are expected to serve as biomaterials for medical use due to the structural similarity to biological soft tissues and thus are expected to have excellent biocompatibility (Figure 1). They are already being studied as a cutting-edge medical material for sutures, artificial blood vessels, artificial skin, cell culturing, hemostatic agents, drug delivery systems and more, and some are advancing to practical application, but from the perspective of longevity, only contact lenses have acquired a large market share.
Figure 1: Hydrogel
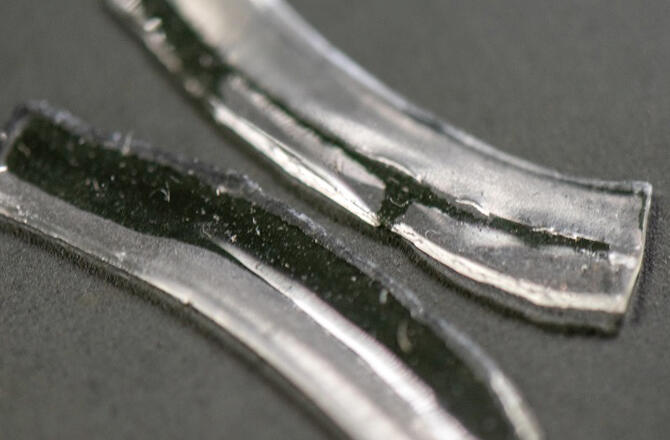
Moreover, plastic materials are currently used for artificial tendons and ligaments, where high strength and durability are required. However, these materials face the issue of fatigue in the body over long periods of time, so new materials are required. "If we can develop a gel that has both strength and durability, we can use it for artificial tendons and ligaments, but gels are brittle, and on top of this, they absorb moisture in the body and swell. They can't maintain their original shape, which was a major challenge," says Professor Takamasa Sakai of the Graduate School of Engineering, the University of Tokyo.
From this background, Professor Sakai established the CREST project "Elucidation of robust-toughening mechanism of gels and development of artificial tendon and ligament," aiming to overcome these challenges and develop artificial tendons and ligaments that are strong, durable, and do not expand in vivo, and is promoting its development as the research director. To achieve the targets - synthesizing polymer gels, carrying out structural analysis and measuring their physical properties and comparing the outcomes of simulation analysis - within CREST, the project is organized into four research groups: theory, synthesis, in-situ measurement and simulation (Figure 2).
Figure 2: CREST structure
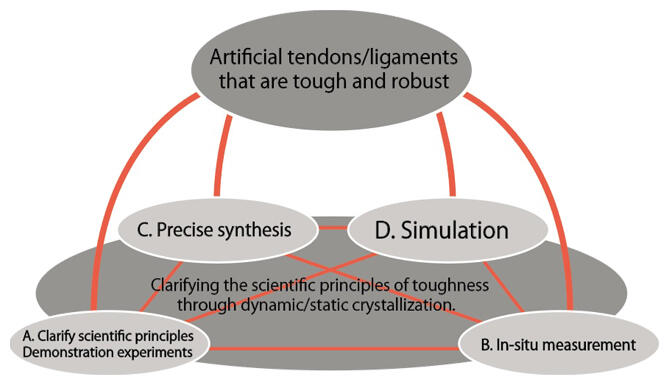
Professor Sakai himself is responsible for clarifying scientific principles and designing and testing gels. In-situ measurement is led by Associate Professor Koichi Mayumi of the Institute for Solid State Physics, the University of Tokyo; Professor Kotaro Satoh of the School of Materials and Chemical Technology, Tokyo Institute of Technology is responsible for precise synthesis; and Professor Yuichi Masabuchi of the Graduate School of Engineering, Nagoya University, is in charge of simulation. "I call this research system 'co-creation via heterogenous groups.' Close cooperation between unique specialists who are active at the forefront of the different fields is leading to results," he says.
The mystery of change in osmotic pressure during gelation clarified for the first time with a universal law
Professor Sakai has a fundamental idea on designing hydrogels for medical use. That is to design materials with consideration of the lifecycle of the hydrogel inside the body. "After embedding the material into the human body, scientists can't alter its properties. Because of this, it is important that we consider more than just formation of gel. During the design stage, we must look at how it will behave in the body, if it will ultimately disintegrate, how many years it can be used, and similar questions," he explains.
Thus, Professor Sakai is once again exploring the fundamentals of gels, having removed conventional preconceived notions. One of his focuses is the change in osmotic pressure that is the basis for water retention capacity. Generally, osmotic pressure indicates the pressure driving the solvent to move from the condensed phase to dilute phase. It is known that it follows the Van't Hoff law of being proportional to the molar concentration of the solute in a dilute solution. On the other hand, it was also known that the osmotic pressure greatly decreases in the process of a liquid-state sol becoming a gel, but the physical laws this process follows were a mystery.
Professor Sakai used tetra gel, for which the polymer network structure can be uniformly controlled, to create static replicas that model the process from sol to gel, and measured the exact osmotic pressure. He found that in the sol state, the osmotic pressure decreased, because the molar concentration decreased as the connectivity between polymers increased, but when the gel is formed, the osmotic pressure became constant, regardless of the connectivity. For the first time in the world, he clarified a universal rule for the gelation process (Figure 3).
Figure 3: The relationship between the gelation process and water retention capacity
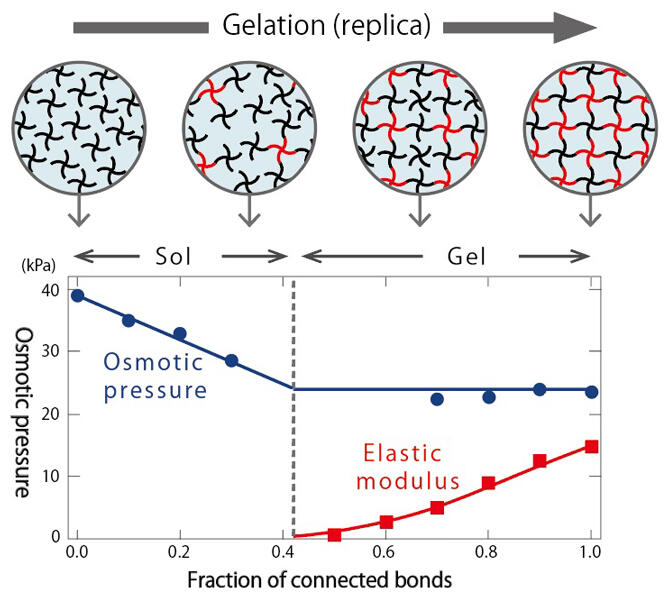
This research is based on the commonality with the phenomenon of a metal becoming magnetic and polymer solutions discovered in 1972 by the French physicist Dr. Pierre-Gilles de Gennes, who won the Nobel Prize in Physics. It has been newly discovered that there is a similar commonality between gels and polymer solutions. This has made it possible to control the water-retention capacity of gels based on a universal mathematical law, and opened the way to develop new soft, wet gels.
Discovering negative energy elasticity and clarifying the temperature change in softness
Next, the group focused on clarifying the physical rules behind the softness of gels. It was believed for almost 100 years that the softness of gels could mainly be explained via entropy elasticity based on the rule of entropy enhancement, the second law of thermodynamics. However, in reality, the deviations in temperature-dependence were observed, so Professor Sakai thoroughly investigated whether the established theory was correct. He designed and created gels with over 50 different types of polymer network structure, measured the temperature-dependence of softness and carried out analysis based on thermodynamics.
Consequently, he discovered, for the first time in the world, that not only entropy elasticity, which ensures that when a gel's shape is altered by an external force the gel attempts to return to its original form, but also another force, i.e. negative energy elasticity is working on the opposite direction at the same time (Figure 4). Professor Sakai emphasized these outcomes: "Until now, gel elasticity was thought to be fundamentally the same as rubber elasticity, but they are substantively different. Moreover, we have learned that the temperature changes were quite a bit larger than conventional understandings - this will surely have a major effect on material development and usage."
Figure 4: The concept of negative energy elasticity
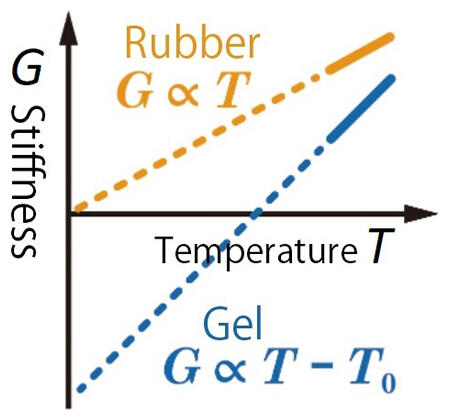
Professor Sakai also focused on clarifying the physics of liquid absorption and swelling of gels, using these outcomes as a base. In everyday life, a phenomenon that hydrogels absorb water and swell is widely utilized - for example, dried wakame (seaweed) and water-absorbing agents in disposable diapers. However, the rule that governs the temperature-dependence of swelling rate wasn't understood. Thus, Professor Sakai made use of the tetra gel and prepared approximately 20 types of gel with independent control of the molar mass of network strand, polymer concentration and network connectivity, then measured the temperature-dependence of swelling rate.
These outcomes led to the world-first discovery that the temperature-dependence of swelling rate is governed by a new rule that combines negative energy elasticity and the Einstein relation in Brownian motion (Figure 5). Professor Sakai commented, "This rule was not apparent just by measuring, but we focused on and analyzed the fact that gel water absorption is due to the random thermal motion of the polymer network (Brownian motion, which explains the random motion of minute particles in a liquid), and so we arrived at a simple rule."
Figure 5: Relationship between the diffusion coefficient of gel (the rate at which the gel swells as it absorbs water) and the elastic modulus (stiffness)
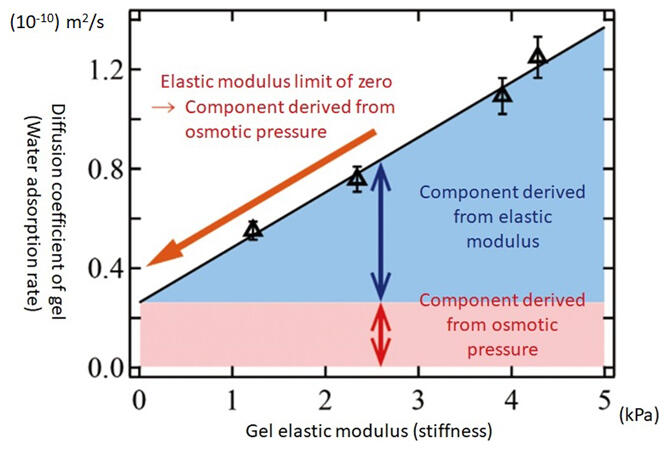
Developing tri-branched gels based on the concept of "less is more"
Stretched by 30 times from the original length
As the group clarified the fundamentals of gels, a student discovered that tri-branched gels can be stretched abnormally. This outcome could not have been predicted from existing theories, so the group repeatedly carried out tests, searching for the reason (Figure 6). Professor Sakai recalls, "Intuitively, I had thought that as the branches decreased the gel would grow weaker, so I was surprised. As we carried out repeated tests, we found that tri-branched gels show a maximum stretch ratio of over 30." (Figure 7 and Figure 8)
Figure 6: Image of tri-branched gel stretching experiment
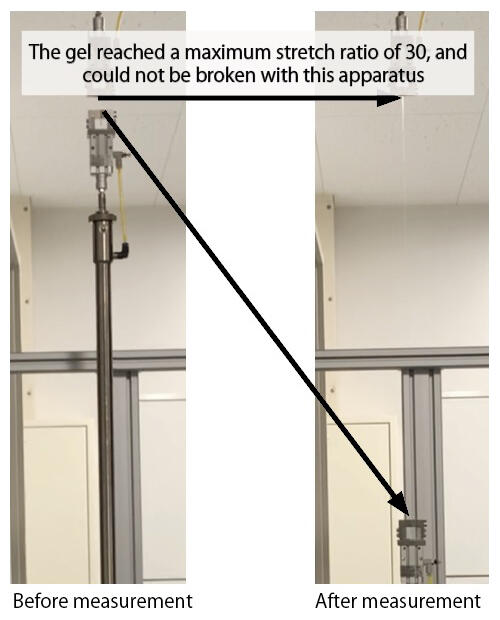
Figure 7: Tri-branched gel stretched close to the theoretical limit
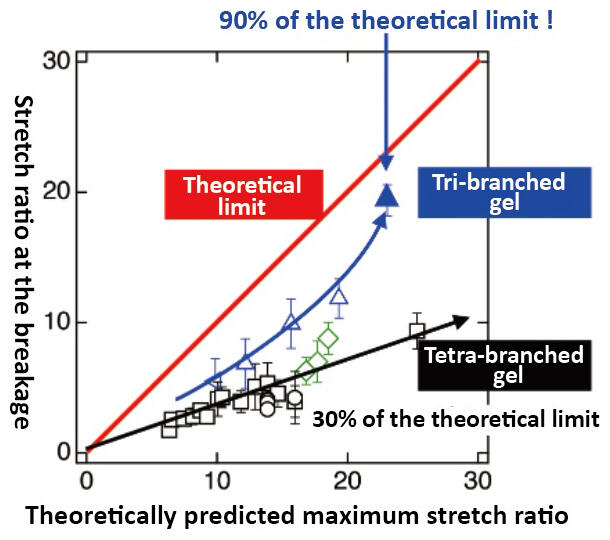
Figure 8: Comparison of the toughness of each branched gel
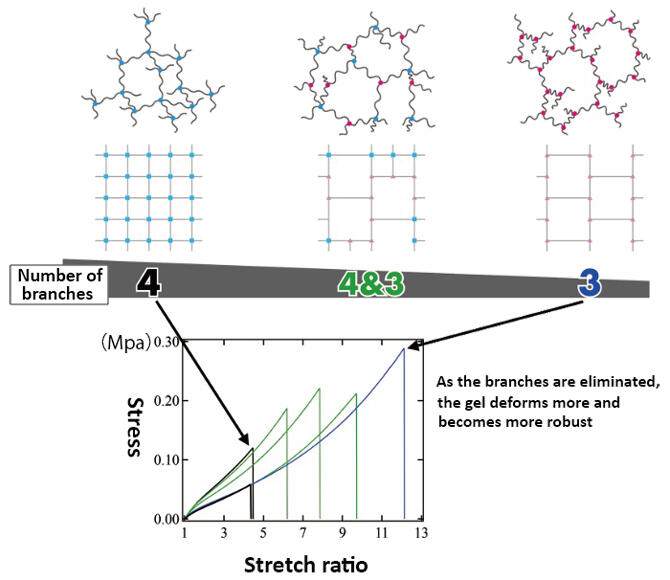
The observation of tri-branched gels using small- and wide-angle x-ray scattering measurement revealed that the excellent toughness is derived from strain-induced crystallization. Strain-induced crystallization is a phenomenon that involves polymer chains that have been highly stretched coming together and forming nano-scale microcrystals. Generally, gels break because force is concentrated in the network's weak spot and small tears (cracks) occur and spread. "Strain-induced crystallization suppresses the occurrence of these cracks and makes the gel tougher. Through experiments, we revealed that they have a robust toughness, always maintaining a certain toughness even when stress was repeatedly added."
In the investigation of tri-branched gels, the group used model PEG gels, which has an easy-to-control well-defined structure, and were able to obtain their desired results in accordance with predictions. However, Professor Sakai points out that they haven't yet developed a ground-breaking gel. "Saying we will produce the outcomes we imagine also means only producing the outcomes we imagine. To create the performance that we want, or performance that surpasses this, we need gels with potential that goes a little beyond theory."
Realizing 10 times the strength of ligaments and tendons
Contributing to the medicine of an era in which humans live for 100 years.
PEG has the issue of lacking crystalline stability in water, so Professor Sakai selected polyvinyl alcohol (PVA), which has higher crystallinity, as a material (Figure 9). PVA has high biocompatibility, and has already been used successfully as a biomaterial, so it is an optimal material for the development of artificial tendons and ligaments. The remaining obstruction is swelling within the body. Swelling refers to hydrogels absorbing water and swelling up inside the body. Swollen hydrogels are unable to maintain their original shape, and lose a great deal of their mechanical characteristics.
Figure 9: The structures of polyethylene glycol (PEG) and polyvinyl alcohol (PVA)
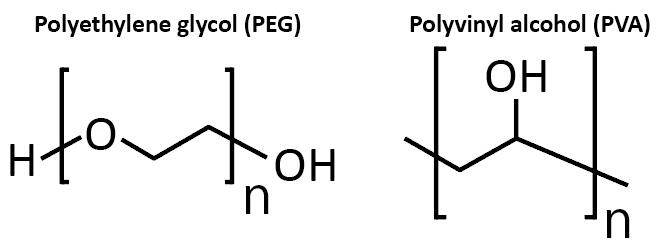
The group succeeded in suppressing the swelling of hydrogel by precisely controlling PVA's crystallinity through network design and the formation process. The gel they created is orientated toward the fiber axis like ligaments, and has high crystallinity.
Professor Sakai talks about his hopes for the future: "We were able to develop a gel with the same or more robustness as we initially expected and confirmed its biosafety. From now on, I want to carefully check whether it can actually be used as a tendon or ligament in the human body. At the same time, it is possible that this material has unprecedented mechanical characteristics, so I also want to explore those further."
As people welcome an age in which they live to 100 years, disability of the apparatus of motion associated with tendons, ligaments and joints has been a major cause of nursing care, and in many cases this damage and functional decline leads to other disabilities. If the group realizes the artificial tendons and ligaments, which substitute the strongest and toughest tissue among biological soft tissues, researchers will see their use in apparatus of motion such as cartilage, meniscus and muscle, as well as for artificial dura matter, blood vessels and skin. It is greatly hoped that these outcomes will lead to increased healthy life expectancy (Figure 10). "We hope to develop our research in the future, not just in medicine but also from the perspective of social issues such as ocean plastic pollution." Professor Sakai will continue moving toward his new goals.
(Text: Kazuyuki Katayanagi; Photo: Hideki Ishihara)
Figure 10: Strength comparison between conventional hydrogels and biological tissues
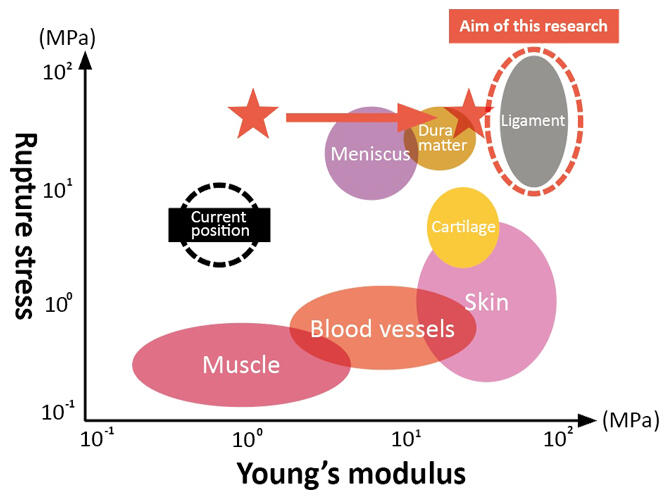